Patterns of Inheritance
We know that a gene is a region of a chromosome (piece of DNA) that
contains a set of instructions for synthesizing a protein (polypeptide). We
know, too that these instructions are passed from cell to cell and from
generation to generation as inheritable traits or characteristics.
We
know that the chromosomes of diploid organisms come in pairs, the homologous
chromosomes, so that each cell of a diploid organism has two genes for each
inheritable trait, one on each of the homologous chromosome pairs.
The
alternative forms (variants) a gene can have on the homologous chromosomes are
called alleles. The precise location where a gene is found on a
chromosome is known as the locus.
A gamete has one of each "gene
pair" (or one of each homologous chromosome) but not both, and that the diploid
number of chromosomes with homologous chromosome pairs, is restored at
fertilization, when two gametes fuse.
At this time we will look at how
genes interact and are expressed in individuals, and how genes are transmitted
from generation to generation.
We did not always know that genes were
located on chromosomes. We did not even know that inheritable traits, or genes,
came in pairs. Gregor Mendel in 1865 was the first to state that
inheritable traits (genes) came in pairs. His work went unappreciated for
several decades because no one seemed to understand what it meant. In the early
1900's other researchers independently made the same conclusions about
inheritance and Mendel's papers were "rediscovered". Soon after, Walter Sutton
showed that Mendel's principles of inheritance applied to chromosomes and that
chromosomes are the units of heredity.
Prior to Mendel, the subject of
inheritance was mostly guesswork. Although the practice of selective animal and
plant breeding was well established, virtually nothing was known of the
mechanisms of inheritance beyond the presence of an egg and a sperm in animals,
and pollen and carpel in plants.
It was generally believed at that time
that characteristics of parents were "blended" in offspring since offspring
generally had features of both parents. No one went so far as to question why,
after several generations, variations still were present, since differences over
time should have been thoroughly blended.
The subject of variation among
individuals and how different variants were passed on (or apparently not passed
on) from generation to generation was very important to science in the 1800's.
Mendel's work coincided with the publications of Darwin and Wallace, who
addressed variation among the individuals of populations as the foundation for
which selective agents could act through time, in the process of
evolution.
Gregor Mendel's Contribution to the Subject of
Inheritance
Mendel observed how specific traits of the garden pea were
transmitted from generation to generation. Mendel kept precise records of the
thousands of offspring (and their characteristics) produced in his crosses. He
then established mathematical probabilities and explanations to validate his
observations.
Although others had studied inheritance, Mendel's
educational experiences in math and observing plant variation helped him design
and analyze his experiments carefully. Mendel:
- Chose a good organism that had a number of "true breeding" traits easy to
observe.
- Designed the experiments carefully. Mendel took plants from true breeding
parents (P generation) , to first generation (F1 hybrids), and then
self-crossed the first generation offspring to form a second generation
(F2).
- Obtained large sample sizes for good data analysis
Mendel's
research led to the following conclusions, two of which are presented as
Mendel's Principles:
Mendel's Statements about Inheritance
There are alternative forms (or variations) of genes, the "units" that
determine inherited traits. The alternative forms of a gene are now called
alleles. To relate this to what we know about homologous chromosomes,
the alleles are located at the same locus on homologous chromosomes.
(Specifically, we inherit the alleles for a gene, not the gene).
An individual will have 2 alleles for each inherited trait. The 2 alleles
may be the same, or they may be different. If the two alleles are the same,
the individual will be homozygous for that trait. If the two alleles
are different, the individual will be heterozygous for the trait.
When the two alleles for a gene pair are different from each other,
one will be expressed, and the second will not affect the organism's
appearance. The allele always expressed is said to be dominant, and the
one that may not be expressed is recessive.
Note: These
statements are true for the traits tested in Mendel's peas and for many genes,
but are not universally true. Many genes have alleles that are equally
expressed, as we shall see, and there are genes that have more than 2 alleles
within the population.
Gametes have just one allele for each trait, because the allele (gene)
pairs are separated (or segregated) during meiosis I when homologous
chromosomes pair and then separate. 50% of the gametes receive one allele and
50% of the gametes receive the alternative allele when the alleles are
heterozygous. (And as Mendel proposed, fertilization results in restoring the
pairs of alleles for the next generation).
This statement ultimately
resulted in Mendel's Principle of Segregation: Pairs of genes segregate
during the formation of gametes (Meiosis), so that each gamete has one of each
gene pair (one allele) but not both. Fertilization restores the gene pairs (on
the homologous chromosomes).
Mendel demonstrated his Principle of
Segregation with many monohybrid crosses, looking at one characteristic
at a time. He could further validate his Principle of Segregation with the
test cross, a cross in which the F1 generation, which
appeared dominant, was crossed to the recessive parent. Their offspring would
exhibit equal proportions of both dominant and recessive forms.
Mendel's experiments with crossing two traits at one time, a dihybrid
cross, resulted in his Principle of Independent Assortment. Each
gene pair is distributed (assorts) independently of other gene pairs into
gametes during meiosis.
We observed this during meiosis when the
homologous pairs of chromosomes align along the equator at metaphase I.
Maternal chromosomes of some pairs align towards one pole some of the time,
and the other pole some of the time. Each meiosis event has a different
alignment pattern.
We know today that each homologous chromosome
pair assorts independently, not specific genes. A gene on one chromosome will
be inherited independently of a gene on a non-homologous chromosome, but all
genes located on one chromosome are inherited as a linkage unit. That is two
genes that are located on the same chromosome will be inherited together, and
not assort independently during meiosis.
Many human traits follow Mendelian inheritance predictions. You will look at
some of those in the laboratory as well as reading about them in your text.
We will not discuss in lecture the specific crosses or predictions which
Mendel did which resulted in his conclusions about inheritance. These crosses
are thoroughly discussed in your text and in the laboratory exercise.
You will be responsible for knowing the following inheritance patterns
and the predicted inheritance ratios for each outside of lecture. (Text
references in parentheses). You will also be responsible for other types of
inheritance patterns that are in your text or discussed in class.
- Monohybrid Cross with dominance (Figure 12-4, p. 216) with a 2nd
generation ratio of 3:1
- Monohybrid Test Cross of heterozygous (p. 217) with an offspring ratio of
1:1
- Dihybrid Cross with dominance (Figure 12-6, p. 218) with a 2nd generation
ratio of 9:3:3:1
- Dihybrid Test Cross of heterozygous with an offspring ratio of
1:1:1:1
Note that Mendelian inheritance predictions follow the
mathematical laws of probability. Although it is fairly "easy" to diagram a
monohybrid test and a dihybrid test using Punnett squares (see figures in text),
making predictions and looking at results for increasing numbers of genes or
other inheritance observations becomes tedious and time consuming. Applying
probability laws is much faster and easier.
Some terms used in
Mendelian Inheritance Tests
True Breeding
- A plant that produces offspring with the same characteristics. The
parental generation is a true-breeding
generation.
Cross Breeding
- A cross between different parental types
- Offspring produced by cross breeding are called
Hybrids
F1 Generation
- The first generation
- Generally first generation offspring are bred among themselves to produce
the second generation. In Mendel's pea plants they
self-fertilized.
F2 Generation
- The second generation
- Mendelian ratios are based on second generation
results
Punnett Square
- A method of visualizing Inheritance crosses
Gene
- The physical unit of heredity; the instructions for producing a specific
characteristic or trait. For example, the characteristic or gene may be flower
color. The alternative forms a gene can have would be the specific flower
colors.
- Since diploid organisms have two sets of chromosomes (the homologous
chromosome pairs), most "genes" are paired, often called the gene
pair
Alleles
The alternative forms or variations a gene can have, such as brown or blue
for eye color, or red or white for flower color. The word trait is often used
to describe the specific alleles, but trait is also used to describe the gene,
too.
A diploid individual will have two alleles for each gene locus.
Within a population there can be more than two alleles for a gene, but
only two alleles will be present in any one diploid
individual.
Locus
- The region on a chromosome where a gene is located.
- The alleles of a gene are located at equivalent places (loci) on the
homologous chromosomes
Homozygous
- The 2 alleles for a gene are the same in an
individual
Heterozygous
- The 2 alleles for a gene are different in an
individual
Dominant Allele (loosely and incorrectly
called a dominant gene)
- An allele which is always expressed, whether it is homozygous or
heterozygous.
- A dominant allele masks or covers the expression of its alternative
allele.
Recessive Allele
An allele which is masked by the presence of its alternative.
A recessive allele will be expressed only when it is homozygous, (when the
dominant allele is absent)
Phenotype
The observable traits of an individual
Genotype
The specific genetic makeup of an individual, or total combination of
alleles present, both those expressed and those not
expressed.
You should also review your knowledge of
homologous chromosomes and the process of meiosis, since the homologous
chromosomes "carry" the alleles, or alternative forms for each
gene.
Beyond Mendel
Mendel's research occurred before we had
knowledge about chromosomes, molecular genetics, mitosis or meiosis. All of
Mendel's genes had dominant and recessive forms, and each of his characteristics
was found on different chromosomes. Early on, some inheritance patterns did not
match the expectations proposed by Mendel's principles. We shall now turn our
attention to some gene actions that go beyond the basic Mendelian
predictions.
The Chromosome Theory of Inheritance
Gregor
Mendel's work was "rediscovered" in 1900 by three independent geneticists who
had done studies which came to the same conclusions that Mendel had made. They
had the advantage that the processes of mitosis and meiosis were known
explaining how genes could be separated. The next step was accomplished in 1902,
when Sutton and Boveri correlated Mendel's conclusions about genes (or inherited
traits) to the behavior of chromosomes during mitosis and meiosis. Sutton is
credited with first proposing the chromosome theory of inheritance:
- Chromosomes are in pairs
- Homologous Chromosomes separate during meiosis so that alleles are
segregated
- Meiotic products have one of each homologous chromosome but not both
- Fertilization restores the pairs of chromosomes
- And -- genes are located on chromosomes
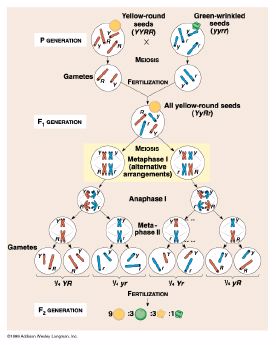
Chromosomes and
Mendel's Laws
Gene Linkage
Today we know that each cell contains several thousand
genes, and that genes are specific regions of chromosomes. Further we know that
entire chromosomes, not individual genes, are transmitted by meiosis to the
gametes. But the inheritance patterns discussed to date have involved genes
located on different chromosomes, so they have followed Mendel's Principle of
Independent Assortment.
We can now look at the inheritance of genes which
are located on the same chromosome, the subject of gene linkage. This
adds some interesting complications to the predicted patterns of inheritance
(and also explains why recombination, which we discussed with meiosis, is so
important as a source of variation).
In 1908, researchers discovered a
dihybrid cross in sweet peas that did not give the predicted Mendelian ratio of
9:3:3:1. They could not explain why their results were closer to 75% and 25%
(the 3:1 ratio expected for a monohybrid cross).
Ultimately it was shown
that the flower color and pollen length (the genes observed) were on the same
chromosome. Since we inherit entire chromosomes rather than independent genes,
all genes on one chromosome are inherited together as a single unit (called the
linkage group), and we should expect a 3:1 inheritance ratio for the linkage
group. This was just the first time someone had seen this.
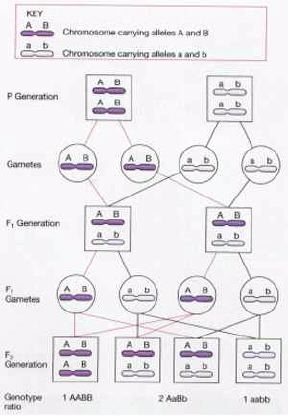
Crossing over results in the exchange of bits and pieces of DNA
between homologous pairs of chromosomes at the chiasmata during prophase I of
meiosis. This process of recombination results in gametes (or meiotic
products) that are not identical; some of the linkage groups have been changed
by the crossing-over. As a result of recombination, new allele combinations are
formed, and we have more genetic variation.
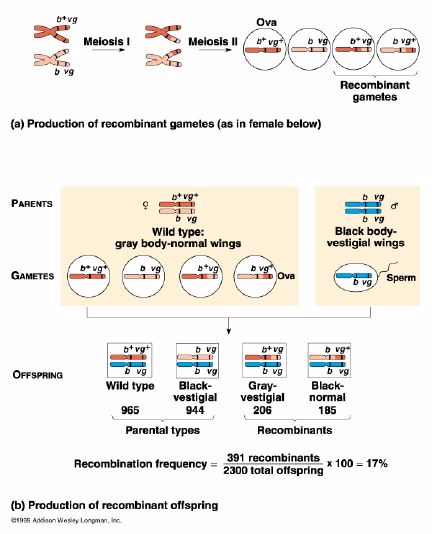
Sex-Linkage and Sex-determining Chromosomes
One of the earliest
discoveries about gene linkage related to another significant thing about
chromosomes and species, especially animal species. By the early 1900's it was
known that males and females of most species have one pair of
"not-exactly-matching" homologous chromosomes, which determined the gender of
the individual. These chromosomes were called the sex chromosomes. (The
truly matching chromosomes are the autosomes.)
With the
gender-determining chromosomes, one sex, usually female, will have two matching
chromosomes (XX) and the other sex will have two unmatched chromosomes (XY). At
meiosis, all eggs will contain an X chromosome, but half the sperm gametes will
have a Y chromosome and the other half will have an X chromosome.
Some
species have the reverse pattern of sex chromosomes (male = XX and female = XY),
and some species have one gender (female) with a pair of chromosomes and one
gender (male) with a single unmatched chromosome. In all cases the gender with
the dissimilar pattern will determine the gender of the offspring.
In
1910, Thomas Hunt Morgan, who spent much of his career studying inheritance
patterns of the fruit fly, Drosophila melanogaster, discovered the
presence of a white eye in certain individuals. Since this was a distinctive
feature, Morgan decided to study the inheritance pattern for this recessive eye
color.
Morgan made several crosses using a white-eyed male, expecting the
standard Mendelian results. He did not get them. While the ratio of 3:1 was
obtained, all of the white-eyed second generation offspring were male flies. All
females had red eyes (and 25% of the males also had red eyes).
Morgan
did a series of reciprocal crosses of white-eye males with red-eye females and
red-eye males with white-eye females. He concluded that the gene for eye color
in the fruit fly was located on the X chromosome. Males passed the trait to
their daughters (on their solitary X chromosome) and mothers passed the trait to
sons. White eyed females could also pass the white eye allele to their
daughters, but if the father fly had red eyes, the eye color of the daughters
would be red, while the eye color of the sons of white-eyed females would always
be white.
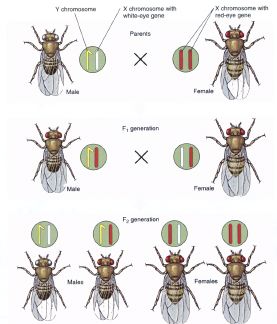
Morgan concluded that eye color was related to sex, and that the
sex-determining chromosomes also had genes that were unrelated to gender
determination. Prior to Morgan's discovery, no one knew that genes unrelated to
gender were also located on these chromosomes.
The other traits are said
to be sex-linked because they are inherited along with the sex of the
individual. Because the X and Y chromosome are not exactly matching, the X
chromosome can have genes that are not located on the Y chromosome, and
vice-versa. Some of these genes are unrelated to the sexual characteristics, but
are inherited with the sex-determination. This is referred to as
sex-linkage.
Some human sex-linked traits are
- Hemophilia (X)
- Hairy ear rims (Y)
- Red-green color blindness (X)
- Duchenne muscular dystrophy
The Barr Body
Revisited
Females have two X-chromosomes. In cells, one of them is
deactivated during embryonic development and forms a tightly condensed object
that lines the nuclear membrane, the so-call Barr body. Transcription does not
occur on the Barr body, a form of gene regulation by chromosome inactivation
discussed earlier. Which X gets condensed for a given cell line appears to be
random. The specific allele for genes carried on the X chromosome that gets
expressed in any given cell line depends on which X chromosome is made into a
Barr body.
The pattern of the calico cat is an example of Barr body
expression. Both orange and black pigment alleles are on the X chromosome. The
black patches of fur are from cell lines where the orange X chromosome is a Barr
body. Orange patches of fur result when the black X chromosome becomes the Barr
body. The patches of white fur are the expression of a different gene.
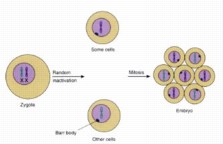
Gene Interactions –
Beyond Mendel
Recall that all of Mendel's genes had dominant and
recessive forms, and that each inheritable trait was found on different
chromosomes. Early on, some inheritance patterns did not match the expectations
proposed by Mendel's principles. We shall now turn our attention to some of the
gene interactions that go beyond the basic Mendelian
predictions.
Single Gene Variations
Lack of
Dominance
Mendel's F1 offspring always resembled the dominant
parent, because each of the genes Mendel chose to study showed complete
dominance. When there is no dominant allele, the heterozygote will have a
phenotype different from either homozygous form. This is sometimes referred to
as an intermediate phenotype.
There are a number of variations in lack of
dominance, but each results in heterozygous conditions that have a phenotype
different from either homozygous phenotype. In other words, when a gene lacks
dominance, there will be three different phenotypes, two homozygous phenotypes
(AA and A'A') and a third heterozygous phenotype (AA').
A. Incomplete
Dominance
- Failure to completely mask the recessive allele
- The heterozygote first generation has some intermediate phenotype between
the two homozygous forms, often a blending of the two alleles.
- Example: snapdragon flowers
B. Co-Dominance
- Both alleles are equally expressed in the heterozygote - both appear in
the heterozygous individuals
- Examples
Roan Cattle (Red X White cows)
Blue Andulusian Fowl (Black
X White)
A-type and B-type Red Blood Cell
Coatings
Lack of Dominance is just one of the many
different ways that genes are expressed.
Multiple Alleles of
One Gene
The "typical" gene has two alleles, one for each of the
homologous chromosomes. This is the same for individuals and within the
population. For some genes, however, there can be more than two alternative
alleles at the single gene locus among members of the species.
- A diploid individual can inherit just two of the possible alleles (one on
each of the homologous chromosomes)
- The effects are shown in the study of the population's variation in
phenotypes for the gene.
- Example: A,B,O alleles for human rbc coats, the "I" gene. The
IA (A) and IB (B) alleles are co-dominant. The i (O)
allele is recessive
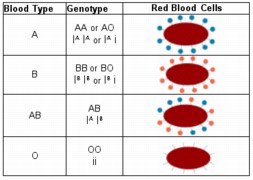
One consequence of the inheritance of the ABO blood type is that the A and B
coatings are antigens, and can trigger antibody reactions in non-complementary
individuals. This is important for blood transfusions, but not in genetics. In
reality, there are variant alleles for each of the blood types beyond those
discussed in biology classes. (See Table 12-1, p.224 for information on
antigen-antibody reactions.)
Interactions Involving More Than One
Gene
Controlling Genes - Epistasis (means standing upon)
- One gene pair controls or alters the expression of other gene
pairs, so that expected phenotypes do not appear.
- Examples:
The gene to distribute pigment can be overridden by a second gene which
blocks (inhibits) pigment production. Mice can have black or brown pigmented
fur depending on the inheritance of a gene for pigmentation. Black is dominant
and brown, recessive. A second, independent gene prevents the distribution of
any pigment in the fur. This gene, when recessive, results in white
mice.
In corn, expression of the pigment gene is also controlled by an
epistatic gene.
Epistasis in Corn Pigmentation
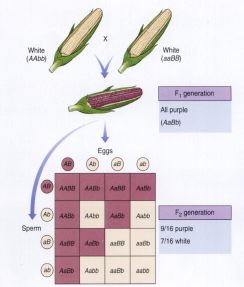
Polygenic Inheritance
The traits that we have so far
discussed all have phenotypes resulting from the interaction of one gene or,
with epistasis, one gene and a second, controlling gene.
Two or more
genes can interact to produce greater numbers of phenotypes. When two or more
genes interact in ways that result in a number of different phenotypes, we see
more variation in the population, with respect to that genetic characteristic.
We call this type of inheritance polygenic inheritance. The individual
phenotype is the result of the combined interaction of all the alleles at all of
the gene loci involved.
Inheritance of Eye Color in
Humans
Human eye color involves the polygenic inheritance of two genes
each of which lacks dominance. The eye color genes code for the production of a
yellow-brown pigment
First Iris Layer Pigment
AA = Produce lots of pigment
Aa = Produce some pigment
aa = Do not
produce pigment
Second Iris Layer Pigment
BB = Produce lots of pigment
Bb = Produce some pigment
bb = Do not
produce pigment
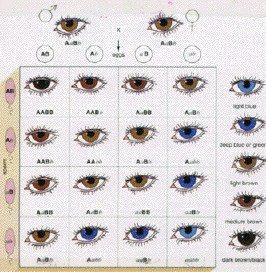
There is a yellow overlay gene which, when combined with the
basic pigment gene alters light brown to hazel and light blue to green.
There are also genes for the distribution of the pigments in the iris
layers, which modify the eye color even more. Such genes have traditionally
been called modifier genes.
Polygenic Inheritance can
also involve two different genes that result in one phenotype.
- The genes may be dominant or lack dominant forms, but in contrast to eye
color, the genes are not identical. When two genes are involved, the
inheritance patten is that of a dihybrid cross.
- Examples
Comb type in chickens
Feather color in parakeets
Kernel color in
wheat
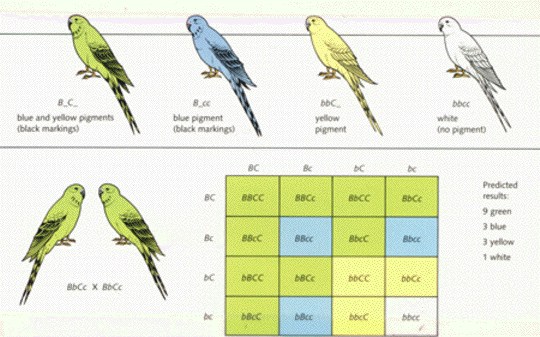
Continuous Variation in Polygenic Inheritance
When several
copies of a gene interact, continuous variation within the population results.
Continuous variation can most easily be demonstrated when population data shows
a bell-shaped distribution pattern when graphed. Skin and hair
pigmentation and height are two examples of such polygenic inheritance in
humans. It is believed that there are at least 3 independent genes, each of
which lacks dominance, responsible for producing the melanin pigment in human
skin (and in hair).
Genes with More Than One Effect - Pleiotropy
(Pleio means "more")
The phenotype conferred by the gene can result in
many additional alterations in the individual, many of which seem unrelated to
each other. In reality, most genes are probably pleiotropic, but some are
dramatically so.
Some Examples
- Albino condition (No Pigment)
- Eye and skin sensitivity to light in many animals
- Frizzle feathers in chickens -- affects feather shape
- Feathers can't insulate properly
- Metabolic problems relating to inability to thermoregulate.
- Abnormal hemoglobin molecule -- affects shape of hemoglobin protein
- Sickle shape rbc
- Metabolic problems
- Malarial resistance
- SRY gene on Y chromosome
Codes for the protein that activates the genes that code for testes
formation. Testes formation activates hormone production that induces
development of other male organs.
- Cystic Fibrosis in Humans -- affects an ion channel protein
- Multiple respiratory problems from mucus blockages
- Blockage of pancreatic ducts
The Influence of the Environment on Gene
Expression
Conditions of the environment can often affect the expression
of a gene. Or stated differently, the environmental conditions can regulate
whether or not a gene gets expressed.
Some examples:
- Temperature-dependent genes
- Some fruit fly wing shapes
- Pigmentation in Siamese cats, and in Himalayan cats and rabbits
- Winter/summer pigmentation in ptarmigan, arctic fox and ermine.
- Morphology of leaves
- Sun/shade leaves
- Submerged/surface leaves in aquatic plants
- Nutrition and Growth
- Height (elongation of bones in growth years)
- Brain development in first two years
- Role of exercise in muscle development
- Sex changes in response to the number of other sex members in the
population (Several species of animals)
Ultimately each
individual is a combination of his/her genetic potential and response to the
multitude of environmental factors to which he/she is
exposed.
Mendelian Inheritance in Humans
How do we apply
Mendelian inheritance to humans? Historically, most of our information about
human genetics, which, like all organisms, follow basic rules of chromosome
inheritance, has come from careful analysis of family histories, or
pedigrees, sometimes over many generations. It is only within the last
generation that advances in molecular genetics have led to much better analyses
of the inheritance of specific genetic traits.
We have been discussing
several different types of gene interactions in this unit, and have given some
examples of human inheritance patterns, such as the inheritance of eye color,
skin pigmentation and the inheritance of multiple alleles with blood typing, and
briefly noted the pleiotropic effects of sickle cell anemia.
At this
time, we will look a bit at how and why we study human inheritance and some of
the things that are occurring in the field of human genetics today. We will also
look at some chromosomal alterations that affect human inheritance. In the next
section, we will also address some of the ways in which biotechnology is
progressing with gene therapy with human genetic disorders.
Most of our
attention focuses on the inheritance of genetic conditions which negatively
impact health and well-being; perhaps because these genetic traits are more
easily identified, and perhaps because we would like to be able to better treat
or prevent these conditions.
Inheritance of Recessive
Alleles
Any mutation has the potential to inhibit the formation of a
needed enzyme. With diploid organisms, however, a mutation most likely affects
just one of the homologues, and the second can still code for the appropriate
enzyme with little or no phenotypic effect on the individual. This has been
demonstrated in laboratory experiments, and is demonstrated with many single
gene inheritance patterns when the heterozygous and homozygous dominant
phenotypes are indistinguishable.
Many of our genetic disorders that
affect metabolism are the result of the inheritance of recessive alleles that
fail to code for the needed enzyme. If this enzyme is critical for survival,
affected individuals, those that are homozygous recessive, will die if they can
not be treated.
It is difficult to remove recessive alleles from the
population since individuals who are heterozygous have the allele but do not
exhibit the problem. In human inheritance, individuals who are heterozygous for
a genetic "disorder", but do not exhibit symptoms are called carriers.
Hence carriers can pass the allele on to the next generation.
In
contrast, it is rare to have serious genetic disorders that are caused by
dominant alleles. The dominant is always expressed so individuals who inherit
the dominant allele express the genetic problem and often succumb to the effects
of the disorder before they can reproduce and pass the trait on to their
children.
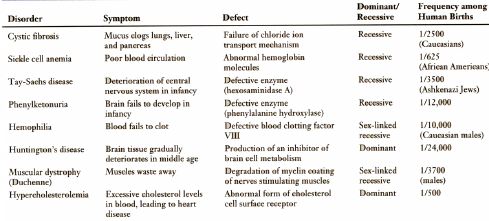
Some
Examples of Human Recessive Alleles which cause problems
Galactosemia
- Inability to convert galactose to glucose in the liver
- Lactose must be avoided to prevent fatal levels of galactose from
accumulating.
Phenylketonuria
- Inability to process the amino acid, phenylalanine
- Protein intake must be critically monitored to prevent buildup of the
amino acid to harmful concentrations. Should be monitored for
life.
Lactose Intolerance
- Inability to breakdown lactose to glucose and galactose
- Avoiding lactose prevents symptomatic problems
Albinism
- Inability to produce any melanin pigment
Hemophilia (This is
also a sex-linked genetic "disorder")
- Inability to produce a critical blood-clotting factor, carried on the X
chromosome
- Hemophilia is now treated by supplying the affected individuals with the
clotting factor. If not treated, hemophilia is usually
fatal.
Tay-Sachs Disease
- Inability to produce critical lipases, which results in accumulations of
ganglioside lipids in the brain. Tay-Sachs is fatal in early
childhood.
Sickle Cell Anemia
- Hemoglobin has a shape which can not pick up oxygen effectively and the
rbcs fracture and block blood flow. This can cause stroke or heart attack, as
well as inhibit circulation in general.
- Treatment by periodic whole blood transfusions
- The partial sickle effect of heterozygotes does not negatively affect
health, and confers resistance to the malaria-causing
protist.
Cystic Fibrosis
- Codes for a membrane protein that functions as a chloride ion channel.
Lack of the chloride ion channel ultimately results in thickened mucus and
excess mucus production, especially in lungs and pancreas. Breathing is
affected, and individuals are very susceptible to infections. Many individuals
die in childhood.
- One treatment today involves gene therapy using genetically altered cold
viruses that contain the normal gene. The viruses are sprayed into the nasal
passages. When the virus invades the nasal mucus producing cells, the gene is
incorporated into the cells and gets transcribed. The treatment is
short-lived, however, since cells are replaced every few weeks.
Some Dominant Allele Problems
As mentioned, those
dominant alleles that negatively impact individuals are rare in the population.
The exceptions are dominant alleles that express themselves post-reproductively,
such as Huntington's disease, which causes the brain to deteriorate, a disease
which affected Woody Guthrie. Although it is possible to identify and screen for
the Huntington's dominant allele, many who have the trait in their pedigree may
choose not to go through the testing procedure. It may be difficult to decide if
one wants to know that he/she will have the symptoms of this brain
disease at "mid-life".
A second dominant "disorder", acondroplastic
dwarfism, is very rare in the human population. This allele is not
lethal.
Chromosome "Abnormalities" and Inheritance
It is fairly
easy to observe our 46 human chromosomes and their shapes, because we can obtain
a karyotype, or chromosome display during the metaphase stage of cell division.
This allows us to see distinct chromosomes, and detect patterns that are not
typical.
For reasons not understood, occasionally, a homologous
chromosome pair will fail to separate during meiosis, resulting in an egg or
sperm with one more or one fewer than the normal complement of chromosomes
(trisomy or monosomy). In general, we call this non-disjunction or
aneuploidy
Most often, a non-disjunction results in a gamete that
does not survive; in some cases, however, some gametes do survive, producing
individuals with abnormal chromosome numbers. Most of these non-disjunctions
have serious genetic consequences. A non-disjunction can affect either the
sex-determining chromosomes or autosomes. We will mention a few human
examples.
Non-disjunction of Autosomes
Survival with an
autosomal non-disjunction is rare.
- Trisomy G or 21 or Down syndrome
As many as 1 in 20 eggs produced after
the age of 40 may carry this chromosome
abnormality.
Characteristics:
Poor muscle tone, including heart
muscle
Tongue and mouth not proportioned, affecting speech
Common mental
retardation
Immune system weak
Non-disjunction of sex
chromosomes
- Monosomy X0
Turner syndrome
Symptoms include absence of secondary
sexual development and sterility. They do not produce Barr bodies.
- Monosomy Y0
Lethal in embryonic development
- Trisomy XXX (female)
No detectable problems. Females are usually
fertile and bear normal XX or XY children. It may be related to the Barr body.
- Trisomy XXY (male) (and other multiples with both X and Y, except
XYY)
Kleinfelter syndrome
Mixed secondary sexual development at puberty
and sterility
Have normal intelligence testing
- Trisomy XYY (male)
Increased vertical stature
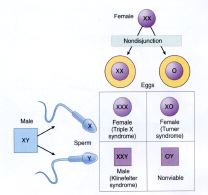
Other Chromosome Abnormalities
Polyploidy
- Increase in the number of sets of chromosomes, usually resulting from the
formation of diploid gametes.
- Occurs naturally in many plants and may produce larger, hardier
individuals.
- If the diploid gamete unites with a normal haploid gamete, the triploid
hybrid is sterile (no homologous matches at meiosis).
- If both gametes are diploid, the individuals are often fertile.
- Polyploidy is used extensively in developing agricultural
varieties.
The impacts of these chromosomal alterations vary,
depending on when and where. In some cases, the cell will not work, and dies. In
gametes, they will be carried in all cell lines, and there is some evidence that
some chromosomal alterations may activate oncogenes, or cancer causing genes.
One form of leukemia is known to be caused by a
translocation.
Extra-nuclear gene expression
And for our final
note on transmission of characteristics from generation to generation, Mendelian
inheritance addresses the behavior of genes on chromosomes.
Organelles,
such as mitochondria and chloroplasts (and all plastids) have small circular
pieces of DNA, and that DNA is transcribed and translated within the organelle.
Mitochondria and chloroplasts are self-replicating. In sexual reproduction, only
the egg cell's cytoplasm is passed to the zygote, so only maternal mitochondrial
and chloroplast DNA will be transmitted from generation to generation. Some
genetic disorders are traced to mutations in mitochondrial DNA that codes for
proteins in the electron transport chain. Mutations in mitochondrial DNA may be
one reason cells age.
Current Research in Genetic Treatments
Before we leave our section
on human genetics, it's good to reinforce where we are in research. Although
this topic overlaps our material on Biotechnology, it makes sense to discuss
what we are doing in research today. Much research takes place in trying to
treat and "cure" genetic abnormalities; for the benefit of the individual and
for humans in general. However, some problems do come up.
A first step in
developing treatments for gene disorders is to know the gene sequence and
location of genes. This has been accomplished with the 12-year Human Genome
project, completed summer, 2000. We can now go forward.
We still have no
permanent cures for genetic diseases. We can treat many genetic diseases and
many research projects are seeking ways to alter the genetic code to repair
faulty genes in affected individuals using cell transplants that carry the
"correct" code.
But most of our treatments of genetic disorders are much
less glamorous than transplanting cells that may survive and produce the needed
substances for survival.
In the past, the genes for many genetic
disorders, which normally would be transmitted to the gametes, were not passed
on, because the genetic "disease" resulted in premature death; the "afflicted"
person never had the opportunity to pass on the problem. As discussed, today,
with better treatment, many such individuals reach maturity. For those
individuals who have genetic disorders, or who carry these traits we need to be
better able to deal with the consequences of the potential to pass on certain
harmful human traits. There are a number of ways to do this
today.
Genetic Screening for early detection of disorders when treatment
can be most helpful
- DNA probes to detect carriers
Cystic fibrosis
Huntington's disease
- Biochemical tests to detect carriers or early detection of disease
Tay-Sachs
PKU (carriers and newborns)
Sickle-cell anemia
- Prenatal analysis --Screening during pregnancy for several abnormalities
(There are risks to doing so, however)
- Amniocentesis to obtain fetal cells in amnionic fluid (14th
to 17th week of pregnancy and several weeks culturing sample)
- Chorionic villus sample of fetal tissue from the placenta
more cells
available than from amniocentesis)and can be done earlier in pregnancy)
- Ultrasound of the developing fetus
- Fetoscopy using a thin viewing scope and fiber optic light source
inserted through the uterus
How do we deal with the results when they indicate a genetic
problem?
Phenotypic treatments
- Diet modification
- If the genetic disorder is one that fails to produce a critical enzyme
for the breakdown of some food or nutrient so that the nutrient accumulates
in toxic quantities, the individual can be treated by developing a diet that
minimizes or does not contain the substance.
- Some examples:
Lactose intolerance
Phenylketonuria (phenylalanine
intolerance)
Galactosemia (galactose intolerance)
- Environmental Adjustments - Avoid the condition which aggravates the
disorder
- Some examples
Sickle-cell anemics avoid excessive oxygen demand situations
Myopic
people wear spectacles
Albinos avoid sun
- Surgery to repair the disorder
- Chemotherapy to inhibit gene activity when the gene produces some harmful
substance or to chelate some chemical that can build to toxic
levels.
Gene Therapy
Gene therapy involves
transplanting cells that contain the "normal" gene into tissues of the affected
individual. To be effective, transplanted cells must:
- not trigger the immune system of the individual to reject them
- must be in the appropriate tissues
- must be functional; that is make the substance the gene codes for in the
appropriate levels.
All of these things are difficult. There
are serious risks when trying to use vectors to splice genes into the
chromosomes. Viral DNA may itself cause problems in the chromosome and
negatively affect gene expression.
We previously mentioned the success
with treating some respiratory symptoms of cystic fibrosis using genetically
altered cold viruses which contain the normal gene.
There has also
been success with transplants of umbilical cord stem cells (a type of white
blood cell) into which the desired DNA has been inserted. Promising, but
controversial, research uses cells of very young embryos and fetal cells,
because such cells often retain their genetic competence and do not trigger
immune rejection in the hosts is ongoing. Some diseases that we hope to treat
with such cells are Parkinson's (transplants into brain tissue) and Diabetes
(cells transplanted into the pancreas). This will be discussed further in our
biotechnology chapter.
Each of us, in our lifetimes, as citizens, may be
making decisions about the use of DNA technology for medicine, food production
and crime, the use of embryonic tissues in research and treatment of diseases,
gene experimentation on humans, and even cloning. These are social, political
and ethical issues. The more knowledge we have about molecular genetics, the
better able each of us will be to make the necessary informed decisions.
